With unravelling the spread of antimicrobial-resistant genes in both urban and agricultural environments, antimicrobial resistance (AMR) is an undeniable fact which threatens public health worldwide. Following the action of the European Union, countries such as China have put the regulation of growth-promoting antibiotics (GPAs) on the priority list. However, if the use of antibiotics in feeds is restricted, it is challenging to run poultry production, the most intensive animal production system, in a cost-effective manner. Therefore, nutritional strategies that can maximize efficiency and food safety, and minimize the use of antibiotics during the rearing process are becoming attractive. The article discusses the role and implications of medium-chain fatty acids (MCFAs, C6:0 – C12:0) in poultry production.
As is known to all, AMR has been a global public health threat for more than a decade, drawing numerous attentions and resources to improve the status quo. Earlier in the 2000s, researchers have evaluated the risks, which outweigh the benefits, of antimicrobial use in animal feeds as growth promotors (Wegener, 2006). At that time, the medical treatment problems that are already attributable to antibiotics in animal feeds, were infections caused by resistant strains of specific pathogens such as enterococci, E. coli, Salmonella, Campylobacter, etc. It remains as today’s problems and gets severer.
What’s in the history
Back in history, with a good intention of combating pathogenic microbes, antimicrobial agents were utilized and improved human health and well-being. For example, antibiotics, as one of the agents, have saved countless lives and enabled the development of modern medicine over the past 70 years. Antimicrobial agents include antiseptics, antibiotics, antifungals, anti-helminthics, etc. (Michael et al., 2014), among which antibiotics have been discovered to improve the growth performance of domestic animals (Moore et al., 1946; Stokstad and Jukes, 1950) and applied in feeds for pigs, poultry and cattle since the 1950s. However, administrating antibiotics extensively and often unnecessarily for the purpose of treatment or prevention of infectious diseases, has provoked an evolutionary response among microorganisms (Sykes, 2010), resulting in increased drug-resistant pathogens in both humans and animals (Czaplewski et al., 2016). A graphic example of AMR is the widespread antibiotic-resistant genes since the introduction of penicillin during World War II. Nowadays, after about 70 years later, the number of pathogens resistant to not only penicillin but to other available antibiotics such as bambermycin has been shown to increase (van den Bogaard et al., 2002). Antibiotics also frequently affect not only a certain pathogenic agent but also the commensal microbiota of the host.
What are the drivers
As stated by the researchers, AMR has been defined as a threat with three main drivers (Michael et al., 2014). Firstly, the frequency of AMR phenotypes among microbes is increasing significantly (Danko et al., 2021). Microbes are able to adapt rapidly and effectively to environmental changes (Gogarten et al., 2002; Janetos, 2009) and have been shown to carry out adaptations to extreme environments such as resistance to heavy metals, UV light, oxidative stress, as well as antibiotic pressure. The higher the selective pressure generated by the antibiotic application, the faster the microbial communities adapt and become resistant to such treatment.
Secondly, the human population nowadays is increasing and well-connected globally. According to the United Nations Department of Economic and Social Affairs (United Nations, 2015), the human population broke through 7 billion in 2015 and will be increasing to over 9 billion in 2050, accompanied by speedy urbanization. Such a large number of individuals living in a compacted environment provides a remarkable opportunity for the rapid proliferation of infectious microbes. This, moreover, projects the need for intensive animal farming to provide sufficient foods, which results in another rapid spread of pathogens among domestic animals. In addition, individuals may travel to most places on the planet within days, contributing to the global distribution of microorganisms, with or without AMR genes.
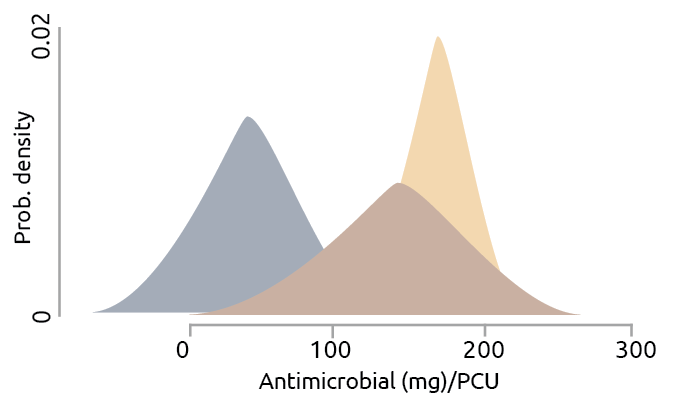
Last but not least, overuse of antibiotics in human and agricultural production is one of the main drivers, which needs the most attention. As estimated, two-thirds of the world’s increase in antimicrobial consumption is due to the growing number of animals raised for food production from 2010 till 2030 (Van Boeckel et al., 2015). Agricultural producers use AGPs to improve the overall health of the stocks and hence increase the ultimate meat/egg/milk yield remarkably. As a result of domestic use of antibiotics, AMR genes become increasingly abundant and diverse in both urban and agricultural environments (Baquero et al., 2008; Nesme et al., 2014; Wright, 2010; Zhu et al., 2013). Among the regression models developed to estimate global antibiotic consumption in animal production, a higher dispersion of the posterior distribution of poultry production, compared to swine production, has been discovered (Van Boeckel et al., 2015; Fig. 1). It suggests that poultry production showed a broadened range of intensity of antibiotic use across regions than swine and cattle production.
Therefore, we must take actions from each sector to raise awareness and effectively improve the AMR situation. In this article, besides the big picture of global AMR as mentioned above, the main focus will be on potential alternatives to growth-promoting antibiotics (GPAs) in poultry production. We will pay special attention to one of the effective candidates, MCFAs, which appear in many scientific studies in recent years.
Alternatives: what are there?
Alternatives to antibiotics are defined by Czaplewski et al. (2016) as non-compound (i.e., non-classical antibacterial compounds) approaches that target bacteria or approaches that target the host to treat bacterial infection. For humans, researchers have been investigating alternatives such as antibodies, probiotics, vaccines, and bacteriophages which are considered promising approaches in the pipeline portfolio (Czaplewski et al., 2016). These alternatives have the potential for animal health as well. However, the anticipated costs for most of them would not facilitate farm animal consumption within years. Besides, MCFAs (Kim and Rhee, 2013; Wang et al., 2015), botanical extracts (Yang et al., 2015), and organic acids (Diebold and Eidelsburger, 2006; Woźniakowska et al., 2017) have been suggested as possible alternatives to classical GPAs in animal feeds.
The challenges: both physiological and technological
Alternating GPAs in animal production is challenging due to the increasing demand for animal products and the complexity of the gastrointestinal (GI) ecosystem, let alone a fundamental understanding of how antibiotics improve feed efficiency is lacking. Possible mode of actions have been proposed (Barug et al., 2006) based on the complexity of the GI environment: 1) GPAs stimulate intestinal synthesis of vitamins by bacteria (Coates et al., 1952; Groschke and Evans, 1950); 2) GPAs reduce total numbers of bacteria in the GI tract and thus a lower microbe-host competition (Dibner and Richards, 2005); 3) GPAs inhibit harmful bacteria which may be mildly pathogenic or toxin-producing (Engberg et al., 2000; Knarreborg et al., 2002; Stutz and Lawton, 1984); 4) GPAs inhibit bacterial urease (Visek, 1978); 5) GPAs improve the energetic efficiency of the gut (Parker, 1990; Sherry et al., 1981); 6) GPAs inhibit bacterial cholyltaurine hydrolase activity (Feighner and Dashkevicz, 1987; Knarreborg et al., 2004); 7) GPAs spare nutrients for host animals (Cunha et al., 1950); 8) GPAs improve nutrient absorption from morphological changes to small intestinal epithelium (Ford and Coates, 1971; Stutz et al., 1983); 9) GPAs modify intestinal enzyme activities (Kawai et al., 1980); 10) GPAs reduce immune stimulation by supressing subclinical microbial challenges on the host (Roura et al., 1992).
Although many studies have attempted to find alternatives to GPAs in animal diets, there is no satisfactory alternative or a “holy grail”. Nonetheless, some products may offer a similar growth benefit and protection against pathogens when applied in combinations to embody the abovementioned multiple modes of action (Allen et al., 2013; Seal et al., 2013). Besides the physiological complexity, unpleasant odor, low solubility, poor chemical stability, and volatile nature of possible alternatives (MCFAs, botanical extracts, and organic acids) evoke even more challenges to the producers to tackle. Combining these alternatives might provide a potential solution to the abovementioned challenges in substituting antibiotics.

Why MCFAs: the antimicrobial fats
The antibacterial effects of fats were discovered in the 1950s when researchers observed a range of fatty acids
inhibited the growth of gram-positive bacteria such as Lactobacillus helveticus (Desbois and Smith, 2010; E.
Kodicek; A.N. Worden, 1945; Galbraith et al., 1971; Hemsworth and Kochan, 1978). Antibacterial fats mainly
contain MCFAs and their monoglycerides of fatty acids, long-chain unsaturated fatty acids (LCUFAs), fatty alcohols,
and long-chain saturated fatty acids (LCSFAs). Their antimicrobial activity can be generally ranked as
shown in Table 1 (Zhou et al., 2019). MCFAs were ranked on the top due to the most rapid and the highest unit
reduction of both virus titer and bacteria count in in vitro culture (see table 1 in Thormar and Hilmarsson, 2007).
MCFAs are with 6 to 12 carbon atoms and straight-chain free fatty acids. Natural MCFAs usually contain an even
number of carbon atoms, including hexanoic acid (C6:0, caproic acid), octanoic acid (C8:0, caprylic acid), decanoic
acid (C10:0, capric acid), and dodecanoic acid (C12:0, lauric acid). The natural MCFAs are from coconut
oil, palm oil, and cuphea oil, among which the coconut oil contains the highest percent of MCFAs, around 60%
(Bhatnagar et al., 2009). The molecular mechanism lies in the amphiphilic chemical structure of MCFAs, which
break through the cell membrane, resulting in the leakage of intracellular materials and bacterial death (Kim and
Rhee, 2013). Figure 2 shows an example of the membrane damage of E. coli O157:H7 caused by a 5-minute
incubation with caprylic acid (A vs. B) in vitro. Interestingly, the deformed structure of the cell membrane was
even more remarkable in the presence of citric acid (A vs. D).
Hovorková et al. (2018) investigated several MCFA-containing plant oils for their antimicrobial activities against
eight pathogenic and commensal bacteria. None of the hydrolyzed plant oils showed any inhibition towards
commensal bacteria, being Bifidobacterium and Lactobacillus spp, at the tested concentrations. As suggested,
the Bifidobacterium resistance can be attributable to the lack of the ferredoxin system. This system is responsible
for reducing the nitro group in metronidazole, leading to higher percentages of Bifidobacterium resistance to
metronidazole (Pélissier et al., 2010). Besides, the negative antibacterial effect on Lactobacillus acidophilus and
Lactobacillus fermentum corresponds to the observations in an earlier study, in which they noted L. helveticus
was not inhibited by a medium containing riboflavin and salt (E. Kodicek; A.N. Worden, 1945). More importantly,
none of the MCFA-containing oils exhibited considerable effect against pathogenic bacteria (MIC80 > 4.5 mg/
ml) before hydrolysis, a process that frees the MCFAs in the oils by enzymes such as porcine pancreatic lipase
(Hovorková et al., 2018).
Apart from the abovementioned studies, other earlier in vitro studies have also demonstrated the antimicrobial
activities of MCFAs on Candida albicans, Pneumococci, Streptococcus, and Micrococci (Bergsson et al., 2001;
Kabara et al., 1972). These microbes are commonly encountered in clinical microbiological specimens of humans
or animals.

of both. (A) Untreated; (B) 1.0 mmol/l; (C)1.0 mmol/l; (D) 1.0 + 1.0 mmol/l (Kim and Rhee, 2013).
Why MCFAs: essential for immunity defense
MCFAs play an important role in the innate immune defense system discovered naturally present in mammalian breast milk, skin, and mucosa, and also induce host defense peptide expression in humans, pigs, chickens, rabbits, and mice. It might be another beneficial supplement to the previously found antimicrobial property of MCFAs. For example, mammalian breast milk contains a large number of antibacterial MCFAs, which mainly are hexanoic acid (C6:0), octanoic acid (C8:0), decanoic acid (C10:0), and dodecanoic acid (C12:0), as well as long-chain unsaturated fatty acids (Decuypere and Dierick, 2003; Smith, 1980). Besides, it was recently discovered that MCFAs induce in vitro gene or protein expressions of host defense peptides (HDPs) in chicken, pig, and human cells, shown in Table 2. HDPs are natural antimicrobials produced by plants, insects, animals, and microorganisms (Sierra et al., 2017), which offer advantages to become useful antimicrobials.

Why MCFAs: growth-promoting effects
It is always important to translate the health-promoting effects into growth efficiency, as it is directly related to the economical sustainability of the poultry farms. After the success of in vitro antimicrobial testing, researchers dived further into in vivo experiments to verify the effects of MCFA supplementation on growth and production performance of the birds.
One of the laying hen studies demonstrated the positive effects of in-feed MCFAs in a 10-week experimental evaluation. The treatment consisted of microencapsulated MCFAs and organic acid blends, with the active ingredients being 1.2% MCFAs, 17% fumaric acid, 13% citric acid, 10% malic acid as the carrier. Four treatments were added with the blend at 0, 0.5, 1, and 2 g/kg respectively in the basal diet, and each treatment group was assigned 6 replications of 6 hens. As result, the egg production was significantly (p<0.001) higher in the 2 g/kg addition group, although there was no difference in egg weight. Besides, the eggshell strength at the end of the trial was significantly (p<0.05) increased in the treatment of 2 g/kg addition (Lee et al., 2015).
For broiler chickens, more attention has been paid to the studies on the growth and carcass performance at the end of 42 days of rearing. Baltić et al. (2019) investigated the effect of Aromabiotic®, a blend of C10, C8, C12, and C6, on growth performance, intestinal morphology, and gut microbiota. Aromabiotic® was added at a rate of 1.6, 1.2, and 1.0 g/kg in starter, grower, and finisher diet respectively for two treatment groups (per group 6 replications of 10 birds), with or without salinomycin as a coccidiostat. In the results, the birds in either treatment group with Aromabiotic® obtained significantly higher (p<0.05) weight gain, as well as for the FCR, at the end of both grower and finisher phases, compared to the birds in control group without any additives. The FCR was reduced by 6.4% by adding Aromabiotic®. There was not much difference in carcass performance but the percentage of the cut of drumsticks with thighs was 0.69% higher (p<0.01) in the Aromabiotic® group than the control group. Besides, MCFAs (Aromabiotic®) supplementation significantly increased the villus length in the duodenal area, which is an indicator of greater enzyme
secretion and better nutrient absorption. This might be one of the reasons for the improved growth efficiency
observed in this study. In regards to the result of gut microbiota, the birds in both treatment groups had
a lower Lactobacillus count than the control broilers, suggesting the modulative effect of dietary MCFAs on
the microbial ecology in a manner similar to coccidiostat (Baltić et al., 2019).
Moreover, another broiler study investigated the dose-response effect of Aromabiotic® on the growth performance
(Khosravinia, 2015). 720 broilers were randomized in 3 groups, where Aromabiotic® was added
at 0, 1.5, or 2 g/kg in the basal diet. It was observed that the inclusion of 2 g/kg Aromabiotic® in the diet
significantly (p<0.001) improved the weight gain of the birds at the end of the grower phase (0 – 21 days),
compared to the control diet. Besides, a significant increase (p<0.05) in the European performance efficiency
index was noted in the birds with 2 g/kg dietary MCFAs mainly due to the reduction in mortality
(p<0.05) compared to the control birds.
Besides MCFAs and nutritional strategies
Although many studies have demonstrated the beneficial effects of all kinds of alternatives such as MCFAs
or blends with certain MCFAs, the general voice is that these products lack consistency and the trial results
vary remarkably from study to study, farm to farm. It calls for further investigations in their modes of action.
Prototyping and optimizing the combination of various alternatives with technological advances in process
should be on the priority list for alternative development. More importantly, coupling the dietary strategies,
good management, and hygiene practices should be encouraged to maximize the flock performance (Aarestrup,
2012; Engster et al., 2002).